The Promise of Terahertz Technology
THz radiations finds unique applications in astronomy, medicine and communications, but not without its own distinct challenges.
The applications of the terahertz (THz) frequency range is stuff of science fiction — T-ray vision, ultrafast wireless, weapons detection — but why isn’t it more common.
The frequency range from 100 GHz to 10 THz constitutes a "gap" in the utilization of the electromagnetic spectrum, separating microwave and optical frequencies, both of which have found extensive commercial use. Terahertz frequencies are often unused. This "no-man's land" of the spectrum offers enormous potential for communications, military, and science, but mastering the physics involved has proven difficult, limiting its use to niche applications or research.
In this article, we will look at the promises of THz technology in various domains of science and engineering.
Specifically, we will cover the potential of THz technology in:
Radio astronomy
Imaging and spectroscopy
6G communications
We will conclude with some engineering challenges.
Read time: 9 mins
THz is also referred to as sub-millimeter wave or far infrared (we will use these terms interchangeably) due to the wavelength of signals being (loosely) between tens of microns and tenths of millimeters. The high frequency and short wavelength of THz signals compared to microwave frequencies provides some unique advantages which have been leveraged in several interesting ways. We will examine some of them.
Radio Astronomy
THz frequencies are attractive for space science applications because half the luminosity of the universe and 98% of the photos emitted since the Big Bang are observable at far infrared wavelengths. However, the earth’s atmosphere is notoriously bad at propagating THz frequencies. The attenuation is so extreme that the signal will attenuate 100 decibels (and become extremely weak) over just 100 meters. This makes the detection of THz waves over the atmosphere impossibly difficult at any reasonably long distances.
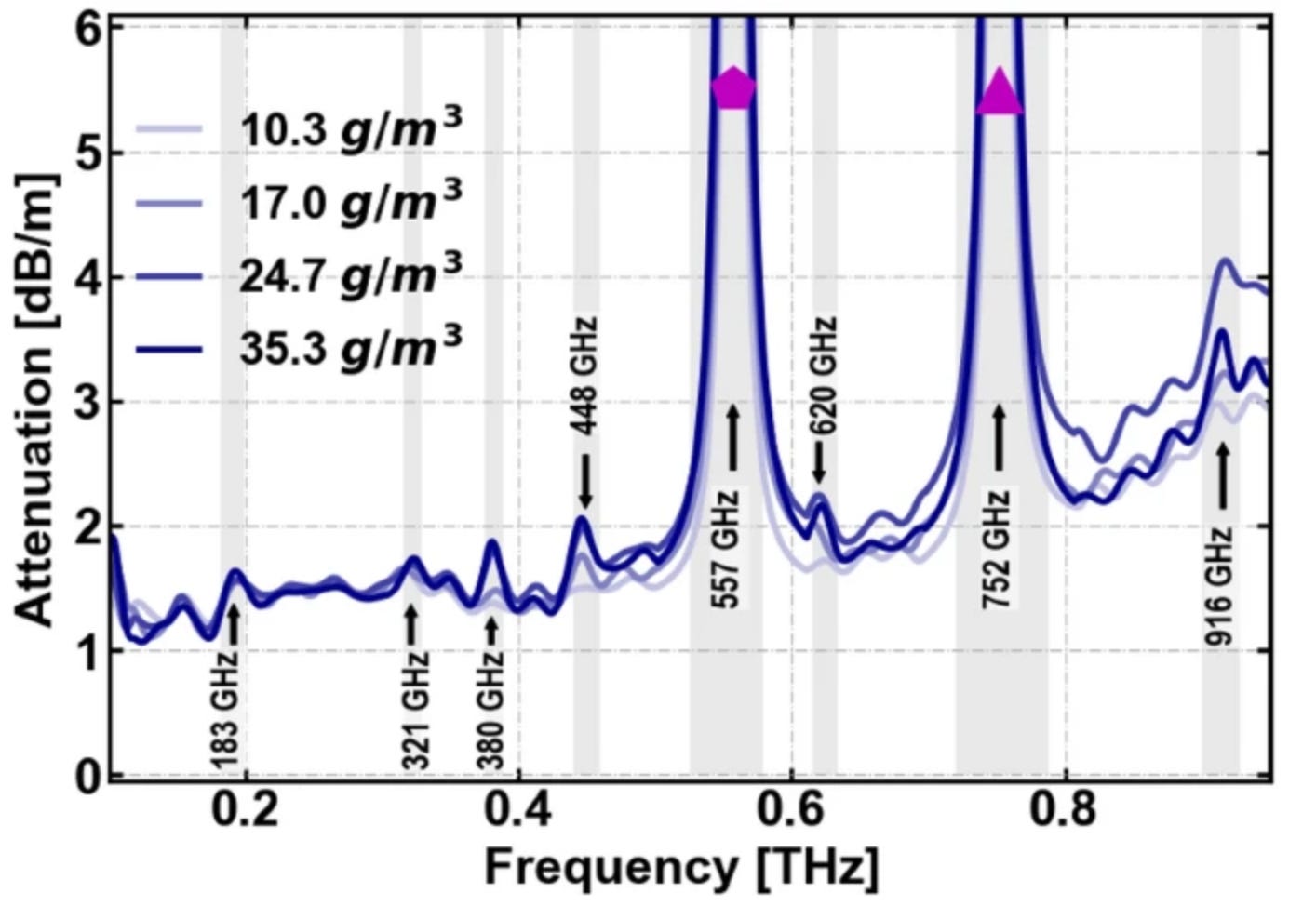
That is, unless you build a radio system that is nothing short of an engineering marvel. While water vapor in the earth’s atmosphere generally prevents observations of space from the surface, precisely engineered observatories utilize specific sub-millimeter wavelengths with low levels of attenuation, at some very remote locations on earth. The ideal location should have a dry, cool atmosphere and be situated away from major centers of electromagnetic pollution. This leaves only a handful of places on earth such as Mauna Kea (Hawaii), Atacama Plateau (Chile), South Pole and Hanle (India). The Atacama Large Millimeter-wave Array (ALMA) in the Chilean desert is the world’s largest ground-based sub-millimeter radio telescope consisting of 66 antennas in an array and costing $1.4 billion to build.
But no atmosphere, no problem — just build observatories in space. Herschel Space Observatory is the largest ever infrared space telescope launched into space and was in operation between 2009 and 2013 (the James Webb1 telescope which was launched in 2021 is now the largest). The Herschel telescope was exclusively built for THz operation, and covered wavelengths of 55-672 microns (0.4—5.4 THz), and has provided some incredible insights.
Data from the Herschel Observatory has led to several important discoveries including the presence of water vapor on dwarf planet Ceres in the asteroid belt, water vapor on Jupiter due to collision of the Shoemaker-Levy comet in 1994, and the presence of molecular oxygen in space. THz astronomy is invaluable from a scientific point of view, and holds the key to the many important discoveries yet to be made.
Imaging and Spectroscopy
The extreme absorption of THz radiation which hinders radio astronomy can instead be leveraged in useful ways for imaging. While the human body is about 60% water, cancerous tumors often have increased vascularity and higher water content due to increased blood supply to infected tissue. At the location of the tumor, there will be greater absorption of THz radiation resulting in a high resolution image of cancerous locations in the body.
THz radiation has difficulty penetrating the human body due to its water content. However, it is beneficial for imaging "surface-level" malignancies in the skin, breasts, or mouth. THz imaging can also be used to measure corneal water content, which can help diagnose eye problems. Alternatively, if you want to avoid wearing heavy lead jackets, THz imaging can be used to detect oral cavities. While we are employing THz radiation to detect water content, another use is imaging burn wounds, where the amount of water in charred tissue is significantly smaller than in its surroundings.
Compared to X-rays, the key attraction to THz imaging is the non-ionizing nature of the radiation. The photons when impinged on human cells have about a millionth of the energy2 in the X-ray spectrum and cannot knock off electrons from biomolecules. This makes the use of THz radiation inherently safer than X-rays, whereas the continued exposure to the latter is damaging to the human body.
Interestingly, nature appears to conceal other mysteries in the THz range. The interactions that occur at the molecular level such as hydrogen bonds, rotations, forces and vibrations produce distinct spectral lines in the THz frequency range. Measuring such spectra with extremely short bursts of THz radiation, a method known as time domain spectroscopy, yields a wealth of information about sub-molecular interactions. The extra benefit is that molecules can be investigated in their natural state, eliminating the requirement for artificial markers or probes that modify the molecule's inherent state.
Future Communications: 6G and Beyond
Monthly mobile data traffic in 2024 is 500 petabytes (0.5 million TB), and it is expected to increase tenfold by the end of the decade. Regardless of the stats, wireless data usage has increased and will continue to do so in the near future. Simply put, we need faster wireless links.
According to the Shannon-Hartley theorem, there are only two ways to improve data rate over a wireless channel.
Increase the signal to noise ratio
Increase the frequency bandwidth
The former is not always easy to do. Increasing transit power comes at the expense of energy consumption, and noise in the radio environment is not in our control. This only leaves option two.
At microwave frequencies, the frequency spectrum is already overcrowded. The table below illustrates the Federal Communications Commission's (FCC) frequency allotment for several radio frequency bands. There is little room for boosting data speeds at microwave frequencies by increasing transmission bandwidth. However, the THz frequency range remains relatively unexplored for communication purposes.
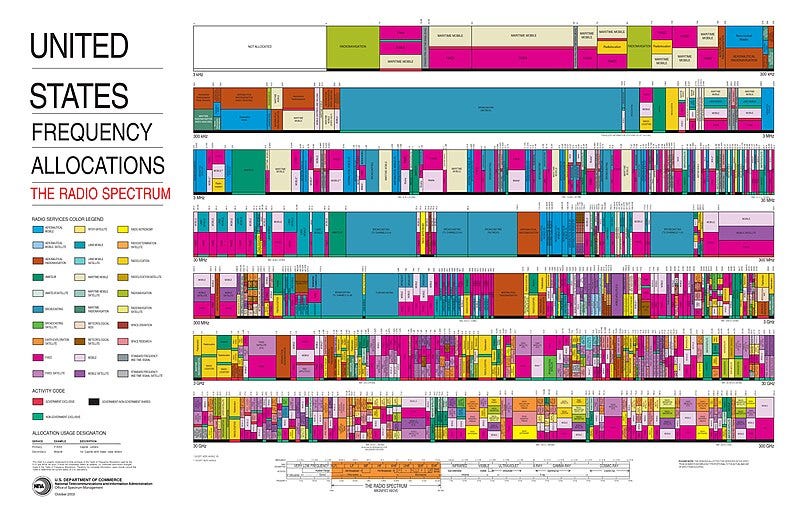
To investigate wireless communications in the THz frequency range, the IEEE 802.15.3d standard was authorized in 2017 and provides a 69 GHz frequency bandwidth from 253-322 GHz. If you look up these frequencies in the previous attenuation chart, you will notice that they represent a region of the spectrum where attenuation is low in the THz range, with no absorption resonances nearby. This careful selection of radio frequency makes THz wireless communications possible. Other frequency ranges for THz communication include D-band (110-170 GHz) and around 400 GHz.
Atmospheric attenuation remains an issue, and THz frequencies will not be effective for long-distance communication. The poor propagation in air is actually a benefit for THz communications since short-range point-to-point lines can be extremely secure. The signal will just not travel far enough to be intercepted.
Because of the short wavelengths, antenna designs for THz radios can be made compact enough for on-chip implementation. However, given to the high attenuation surroundings involved, the antennas must be very directional and utilize THz phased antenna arrays. Researchers have already demonstrated a D-band 8 element phased array in RF SOI that holds potential for THz communications.
It is easy to imagine the benefits of ultra-high-speed links — instant video transfers, lag-free, high-resolution wireless links, wireless backhaul to replace fiber optics, wireless data center links, high-speed inter-satellite mesh links — some articles even envision high-resolution holographic representations and "digital twins". As a society, we have always found applications for faster connectivity, as seen by multiple generations of communication technologies.
But why don't we have THz technology yet?
The Problem of THz Power
Many of the characteristics of THz radiation have been recognized for a long time. It has not received widespread adoption because of a lack of appropriate power sources at these frequencies.
Amplification is required to generate power, and the majority of microwave amplifiers use transistors. Fmax is a technology-agnostic metric that measures a transistor's ability to deliver amplification. Beyond this threshold frequency, transistors can no longer amplify signals. Most microwave designers prefer to operate at frequencies lower than fmax/2, where the transistor provides sufficient gain.
However, most cutting-edge commercially available transistors in mass-production today have fmax values in the hundreds of GHz range, implying that the best transistors available can barely amplify THz signals to provide any respectable level of transmit power. And, given the significant attenuation traits of THz signals, we require a large amount of power.
The most promising candidates today for power generation at THz frequencies are III-V technologies such as Gallium Nitride (GaN), Gallium Arsenide (GaAs) and Indium Phosphide (InP) technologies. An InP transistor has been recently demonstrated to have an fmax of > 1 THz owing to the excellent electron transport in these heterojunction materials. In 2015, Northrup Grumman demonstrated the first InP-based amplifier that provides about 9 dB of amplification at 1 THz.
Over the last decade, substantial advances have been made in THz power generation, which is still an active area of research today. It may be tempting to believe that technologies that do not operate entirely on silicon would never reach the mainstream market. This would be an incorrect conclusion.
Almost all mobile communication devices continue to employ Gallium Arsenide for power amplification because silicon has failed to meet performance expectations. THz electronics may also be a collection of disparate technologies bundled on a module. It appears that is just how the RF world works3.
References
[1] D. T. Leisawitz et al., “Scientific motivation and technology requirements for the SPIRIT and SPECS far-infrared/submillimeter space interferometers,” presented at the Astronomical Telescopes and Instrumentation, J. B. Breckinridge and P. Jakobsen, Eds., Munich, Germany, Jul. 2000, pp. 36–46. doi: 10.1117/12.393957.
[2] A. Y. Pawar, D. D. Sonawane, K. B. Erande, and D. V. Derle, “Terahertz technology and its applications,” Drug Invention Today, vol. 5, no. 2, pp. 157–163, Jun. 2013, doi: 10.1016/j.dit.2013.03.009.
[3] S. Thomas, J. S. Virdi, A. Babakhani, and I. P. Roberts, “A Survey on Advancements in THz Technology for 6G: Systems, Circuits, Antennas, and Experiments,” Jul. 02, 2024, arXiv: arXiv:2407.01957. Available: http://arxiv.org/abs/2407.01957
If you like this post, please click ❤️ on Substack, subscribe to the publication, and tell someone if you like it. 🙏🏽
If you enjoyed this issue, reply to the email and let me know your thoughts, or leave a comment on this post.
We have a community of RF professionals, enthusiasts and students in our Discord server where we chat all things RF. Join us!
The views, thoughts, and opinions expressed in this newsletter are solely mine; they do not reflect the views or positions of my employer or any entities I am affiliated with. The content provided is for informational purposes only and does not constitute professional or investment advice.
James Webb telescope does not have a far infrared detector, so it is not technically a THz radio telescope. The longest wavelength it supports is 28 microns, or about 10 THz in frequency. The module that performs the detection is classified as a mid-infrared detector.
Photon energy is directly proportional to frequency, or inversely proportional to wavelength. E=hf, where h is Planck’s constant, E is the photon energy and f is frequency.
The digital world is just entering the chiplet era for the same reason that one technology node cannot always deliver on price and performance.
Thanks for sharing. It's surprising to know that the Herschel telescope could cover up to 5.4 THz. I thought the THz range was still in research and not yet in real-life applications. By the way, do you have any idea how modeling is done for III-V devices? Do they use the same method as BSIM for CMOS?